In the previous millennium, our group’s research focused on a variety of paleobiological and paleoceanographic topics. Specific projects focused on:the structure and dynamics of ancient ecosystems (including causes and consequences of the end-Cretaceous mass extinction),
- the global distribution of marine zooplankton,
- the biological role of alkenones and causes of non-thermal variation in their saturation (a common paleotemperature proxy),
- the structure of the ocean-climate system in the “greenhouse” late Cretaceous (67 million years ago and 83 million years ago),
- the evolution of the ocean-climate system in the “icehouse” Pleistocene (0-3 million years ago),
- major impact events of the last 65 million years.
Dynamics of ancient ecosystems (including consequences of end-Cretaceous mass extinction)
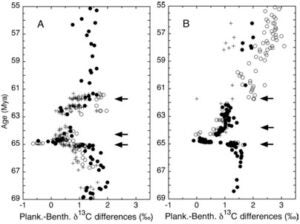
Members of the D’Hondt laboratory have extensively studied the influence of major biological events (extinctions and radiations) on ecosystem structure and the relevance of metabolic strategies for major evolutionary radiations and survival of major extinctions.
Our earliest studies in this area principally relied on fossil data. One of these studies showed that open-ocean planktonic foraminiferal populations in the Atlantic, Pacific and Tethyan oceans change completely at the stratigraphic level of the end-Cretaceous impact debris (D’Hondt and Keller, 1991, Marine Micropaleontology). Another study confirmed that planktonic foraminifera with widely divergent morphologies evolved from a common ancestor very quickly after the end-Cretaceous mass extinction (D’Hondt, 1991, Journal of Foraminiferal Research).
We used isotopic and sedimentological data to show that the marine ecosystem and the biogeochemical cycling of carbon did not fully recover from the impact and mass extinction for more than three million years [D’Hondt et al., 1996, GSA Special Paper 307 and 1998, Science; Adams et al., 2005, Paleoceanography; D’Hondt, 2005, Annual Review of Ecology, Evolution and Systematics (AREES); Coxall et al, 2006, Geology]. We hypothesized that the long delay in recovery of carbon cycling resulted from altered ecological structure in the post-extinction ocean. Final recovery of planktonic foraminiferal diversity immediately followed final recovery of the carbon cycle (Coxall et al., 2006). This diversification may have resulted from the reappearance of oligotrophic oceans as the organic flux from the surface ocean to deep water fully recovered from the mass extinction. Other studies used Milankovitch-scale sedimentary cycles to show that sedimentation rates declined drastically at the time of impact (Herbert and D’Hondt, 1990, EPSL) and that the oceanic response to Milankovitch-scale climate forcing was altered for a million years after the impact (D’Hondt et al., 1996, Geology).
In other studies, we used stable isotopes of carbon and oxygen to document (1) the occurrence of photosymbiosis among Cretaceous and Paleocene species of planktic foraminifera (D’Hondt and Zachos, 1993, Paleoceanography; D’Hondt et al., 1994, Paleobiology; D’Hondt and Zachos, 1998, Paleobiology) and (2) the role of photosymbiosis in the early Cenozoic radiation of planktonic foraminifera (D’Hondt, 2005; Coxall et al., 2006).
Global distribution of marine plankton
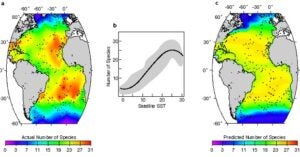
We used the Brown University Foraminiferal Data Base and oceanographic data to map the geographic distribution of planktonic foraminiferal species and to quantitatively test hypotheses of the factors that control variation in species richness (Rutherford et al., 1999, Nature). Our analysis showed that sea surface temperature measured by satellite explains nearly 90 percent of the geographic variation in planktonic foraminiferal diversity throughout the Atlantic Ocean. Temperatures at standard water depths (50, 100, and 150 meters) explain the diversity pattern nearly as well. These findings indicate that geographic variation in zooplankton diversity may be directly controlled by the physical structure of the near-surface ocean. Our results further showed that planktonic foraminiferal diversity does not strictly adhere to the traditional paradigm of continually decreasing diversity from equator to pole. Instead, it peaks in the middle latitudes in all oceans.
Biological role of alkenones and their saturation variability in Emiliania huxleyi
The ratio of 37-carbon diunsaturated to diunsaturated and triunsaturated alkenones (UK’37) produced by some haptophytes is widely used as a proxy for past sea surface temperatures. However, our isothermal culturing experiments with Emiliania huxleyi show that UK’37 values vary with nutrient availability and cell division rate (Epstein et al., 1998, Paleoceanography). These results provide a reasonable explanation for large isothermal variation in UK’37 values of single coccolithophorid strains grown in culture. They also suggest that alkenone-based estimates of past sea surface temperatures may have been influenced by dissolved nutrient concentrations as well as by temperature.
The biological function of C37 alkenones and, consequently, the cause of their temperature-dependent saturation, was previously unknown. In order to assess their cellular role, we cultured strains of E. huxleyi at 12:12 and 0:24 light/dark cycles (Epstein et al., 2001, Organic Geochemistry). Alkenone concentrations generally increased through both logarithmic and stationary phase in cultures with 12:12 light/dark cycles. They decreased when E. huxleyi was energy-deprived (in continuous darkness). These patterns of increasing concentration when light is available and decreasing concentration in darkness are typical of metabolic storage molecules in cultures of other marine phytoplankton. These results suggest that Emiliania huxleyi uses alkenones for metabolic storage. If C37 alkenones are primarily used for metabolic storage, the temperature dependence of their unsaturation may result from differences in melting point, density, or enzymatic optima of biochemical pathways of the differently saturated alkenones.
Causes of end-Cretaceous mass extinction
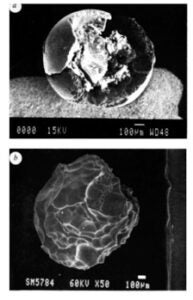
Laboratories throughout the world have accumulated a tremendous amount of evidence that the end-Cretaceous extinction was ultimately caused by the impact of a large extraterrestrial object. The first compelling evidence for this extraordinary hypothesis was provided by Alvarez et al. (1980, Science). Many proximate causes have since been proposed to link the impact to the extinction, including global darkness, acid rain and worldwide wildfire.
Our research group contributed to this area of study in several ways. We were the first to discover impact glass at the event horizon (Sigurdsson et al., 1991a, Nature). We linked the composition of that glass to the principal lithologies of the then recently discovered Chicxulub (Mexico) feature (Sigurdsson et al., 1991b, Nature). Thanks to the work of many laboratories, that feature is now widely recognized as the site of the end-Cretaceous impact. We were among the first to recognize that the unusually sulfur-rich nature of the Chicxulub target may have rendered the impact unusually devastating (Sigurdsson et al., 1992, EPSL). We then quantified the potential environmental effects of sulfuric and nitric acids generated by the impact and assessed their potential biological consequences (D’Hondt et al., 1994, Geology).
Schultz and D’Hondt (1996, Geology) recognized that geophysical signatures of the Chicxulub impact structure closely resemble asymmetries produced by experime
ntal oblique impacts and recognized on planetary surfaces. These signatures suggest that the impact occurred from the southeast at an angle of 20–30°. Consequently, extinction may have been most rapid and severe in the Northern Hemisphere. Geographic variation in floral extinction and recovery is consistent with the proposed trajectory.
For brief reviews of the evidence for the end-Cretaceous impact, its relation to mass extinction, and proximate causes of extinction, see D’Hondt (1994, in Extinction and the Fossil Record) and D’Hondt (2005, AREES).
Structure and evolution of the ocean-climate system
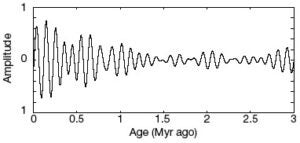
Our studies of the past ocean-climate system principally focused on two topics. The first topic is orbital modulation of the ocean and climate. These studies include the first demonstration of semi-precessional modulation of the ocean-climate system (Park et al., 1993, Science), the above-mentioned recognition that Milankovitch-scale climate forcing was altered for a million years after the end-Cretaceous impact (D’Hondt et al., 1996, Geology), and the inference that strengthening of the semi-precessional cycle in the northern hemisphere triggered the transition to sustained 100,000-year glacial cycles 1.5 million years ago (Rutherford and D’Hondt, 2000, Nature).
The second topic is the physical structure of the late Cretaceous (~67 Ma) ocean. In these studies, we used oxygen isotopic data to infer that (1) the equator to pole sea-surface temperature gradient was lower than that it is today (D’Hondt and Arthur, 1996, Science), (2) the tropical sea surface may have been cooler then than it is today and was probably no warmer (D’Hondt and Arthur, 1996, Science), (3) there were at least three deep watermasses at that time, ranging in temperature between 5-7°C and 13-15°C (D’Hondt and Arthur, 2002, Paleoceanography), and (4) these watermasses originated principally in the Southern Ocean and the North Atlantic, much like the cooler deep waters of today (D’Hondt and Arthur, 2002, Paleoceanography). The second of these four results was challenged by Pearson et al. (2001, Nature), who inferred that the late Maastrichtian tropical sea surface was as warm as that of today or warmer.